Antimicrobial agents have changed human and animal health systems by revolutionizing our weaponry in the war against infectious diseases, resulting in improved survivability for both humans and animals. However, this health triumph has been tempered by the subsequent realization that bacterial populations can quickly modify themselves to resist antimicrobial agents, propagate these resistance traits, and even share resistance genes with other bacteria within their environment. Recently, these abilities have seriously compromised the usefulness of antibiotics in the war against microbes and have created concerns over a future when antimicrobials may have limited usefulness in the management and control of microbial infections.1
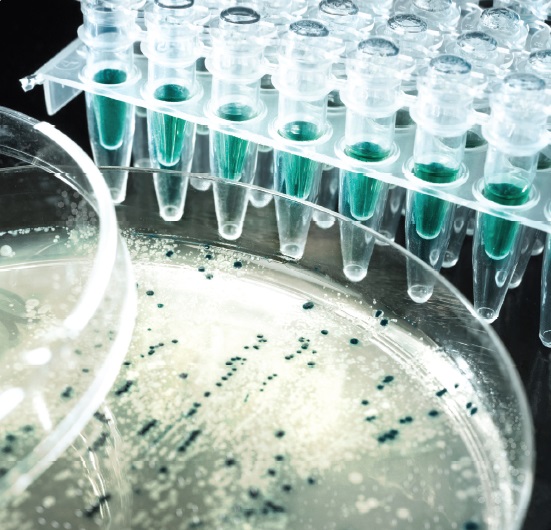
Resistance is a condition in which an antibiotic agent fails to harm a pathogen enough to manage or cure an infection or disease.2 The resistant bacteria have mutated and adapted to their changed environment by the normal evolutionary processes of living organisms. Bacteria, including human pathogens, have developed defense mechanisms for protection against human-produced poisons (ie, antibiotics).3 Bacteria can develop resistance to antibiotics by various mechanisms including the mutation of existing genes4,5 or the acquisition or sharing of genes from other strains or species.6,7
The sharing of genes between bacteria by horizontal gene transfer occurs by many different mechanisms. Mobile genetic elements, including plasmids, phages, and transposons, mediate the exchange of resistance genes. In other circumstances, the presence of low levels of an antibiotic in the environment promotes gene transfer,8 perhaps ensuring that a whole microbial community develops protection against these killer agents.9
The rapid development of antibiotic resistance has led to a continual need to develop new antibiotics, but in recent years, the pace of antibiotic development has slowed, creating concerns that some microbes may gain the upper hand when they cause infections.10
MECHANISMS OF RESISTANCE
Drug Degradation or Inhibition
One mechanism by which bacteria have gained resistance against certain kinds of antibiotics is through the secretion of enzymes that degrade and inhibit the drugs' bacteriostatic and bactericidal properties. Antibiotic resistance coevolved with biosynthesis as a means of bacterial self-immunity strategies for the production of toxic secondary metabolites in antibiotic-producing bacteria. This coevolution strategy could have independently evolved de novo in nonproducing organisms or could be imported via horizontal gene transfer. The genes for resistance, stably integrated into the genome under selective pressure, reflect prior exposure during the evolution of the species.11
Enzymatic degradation of antibacterial drugs is, perhaps, the major mechanism of bacterial resistance to antimicrobial agents of natural origin. Bacteria have been using this mechanism to resist β-lactam antibiotics by using penicillinases, cephalosporinases, and metallo-β-lactamases. Bacteria have also inhibited aminoglycosides through N-acylases, O-nucleotidylases, O-phosphorylases, adenylases, and aminocyclitol. Bacteria even degrade chloramphenicol and fusidic acid by way of chloramphenicol acetyltransferase. Lastly, fosfomycin and macrolide-lincosamide-streptogramins are resisted by bacteria with macrolide esterases and macrolide phosphotransferases.12
A common example of this mechanism of resistance formation is the hydrolytic deactivation of the β-lactam ring in penicillins and cephalosporins by the bacterial enzyme β-lactamase. The inactivated penicilloic acid is then ineffective in binding to penicillin-binding proteins (PBPs), thereby protecting the process of cell-wall synthesis. This strategy has also been observed in Enterobacteriaceae against chloramphenicol in a process called acetylation and in Gram-negative and Gram-positive bacteria against aminoglycosides through phosphorylation, adenylation, and acetylation.1
Alteration of Bacterial Proteins
Bacterial proteins are common targets of antimicrobials. The alteration of bacterial proteins has become a widely used drug resistance mechanism for bacteria. This is one of the three major mechanisms of resistance, along with reduction of drug permeability to its target and drug modification.
Resistance by the general mechanism of drug target modification can be brought about by a remarkable variety of means, which have been exploited by different clinically important bacteria. The modification mechanism often results in an alteration of the original drug target structure, so that the drug binds poorly or not at all. This change in the structure can be brought about by naturally occurring spontaneous mutations in the gene or genes encoding the drug target. These mutations result in modification of single or limited sequences of amino acids in the target protein, often in the region of a known putative drug binding site.11
Examples of this mechanism include quinolone resistance due to alterations in target enzymes DNA gyrase and topoisomerase IV involved in DNA synthesis, rifampicin resistance due to alterations in the β-subunit of the target RNA polymerase involved in RNA synthesis, and low-level penicillin resistance in Streptococcus pneumoniae due to alterations in the transpetidases (PBPs) involved in cell-wall synthesis.13
More extensive modifications of drug targets often require other genetic mechanisms. In the case of high-level penicillin resistance of S pneumoniae, more extensive modifications of the target transpeptidases involved in cell-wall synthesis are possible because of this organism's ability to exchange DNA segments with related bacterial species, some of which have transpeptidases that bind penicillin poorly, allowing the generation of mosaic transpeptidases with extensively modified regions of these target enzymes in S pneumoniae.11
In other cases, such as glycopeptide resistance in enterococci and macrolide resistance in many bacteria, the target structures to which these drugs bind, specifically the cell wall in glycopeptides and the bacterial ribosome in macrolides, are exogenously modified by enzymes encoded by DNA acquired on mobile genetic elements, such as plasmids and transposons, which can be transferred between bacteria. In other cases, such as tetracycline resistance in many bacteria and plasmid-encoded quinolone resistance due to Qnr proteins in enteric Gram-negative bacteria, the drug targets are protected from drug action but not modified by the resistance-determining proteins.6
Altered Metabolic Pathway
Another novel variation of the altered-target mechanism is overexpression of unmodified drug target binding sites in such a way that binding of drug to these extra sites limits access of the drug to a subset of critical target binding sites. This is thought to be the cause of low-level glycopeptide resistance in staphylococci.
Finally, in a number of cases, such as resistance to methicillin and other β-lactams in staphylococci, resistance to mupirocin in staphylococci, and resistance to trimethoprim in many species, bacteria have acquired genes. Sometimes on mobile genetic elements, these acquired genes encode an alternative or bypass drug-resistant target enzyme. This enzyme then provides the functions that would otherwise have been inhibited by the drug, allowing growth in the presence of the antimicrobial.13
Some resistant bacteria evade antimicrobials by reprogramming or camouflaging critical target sites to avoid recognition. Therefore, regardless of the presence of an intact and active antimicrobial compound, no binding or inhibition takes place. This type of evasion has been observed in staphylococci against methicillin and other β-lactams, specifically through changes or acquisition of different PBPs that do not sufficiently bind β-lactams to inhibit cell-wall synthesis. Enterococci are able to resist vancomycin by the alteration in cell-wall precursor components to decrease binding of vancomycin. Mycobacterium spp. effectively use this type of mechanism for resistance against streptomycin through modification of ribosomal proteins or rRNA, against the rifamycins through mutations in RNA polymerase, and against the quinolones through mutations in DNA gyrase.1
Thus, the creativity of nature in developing resistance mechanisms under selective pressure has been capable of meeting the many challenges posed by the development of new antimicrobial drugs.
DRUG PENETRATION PATHWAYS
Most antibiotics target intracellular processes, and activity is achieved by penetration of the molecule into the bacteria. The outer membrane of Gram-negative bacteria provides a naturally occurring shield that becomes an additional barrier to molecular penetration of antibiotics.
For these bacterial entities, there are essentially two pathways that allow penetration through the outer membrane. The first mechanism is a lipid-mediated pathway for antibiotics with hydrophobic molecules; the second is via porins that allow general diffusion of hydrophilic antibiotics. Bacterial sensitivity to molecules then becomes a factor of particular lipid and protein compositions of the outer membrane. Drug resistance involving modifications of these lipid and protein macromolecules is common.14
In this dual capacity, the outer membrane emerges as a sophisticated macromolecular assembly, the complexity of which has been unraveled only in recent years. By combining a highly hydrophobic lipid bilayer with pore-forming proteins with specific size-exclusion properties, the outer membrane acts as a very selective barrier. The permeability properties of this barrier have a major impact on the susceptibility of the microorganism to antibiotics, which are essentially targeting intracellular processes. Small hydrophilic drugs, such as β-lactams, use the pore-forming porins to gain access to the cell interior, while macrolides and other hydrophobic drugs diffuse across the lipid bilayer. The existence of drug-resistant strains in a large number of bacterial species due to subtle lipid or protein modifications in the composition of the outer membrane highlights the importance of the outer membrane barrier in antibiotic sensitivity.15
Porins provide a pathway through the outer membrane for hydrophilic antibiotics, such as β-lactams, as well as tetracycline, chloramphenicol, and fluoroquinolones.16 Any decrease in the ability or rate of entry of these compounds can lead to resistance. There is an abundance of reports of antibiotic resistance acquired through loss or functional change of porins in a large number of organisms, such as Escherichia coli, Pseudomonas aeruginosa, Neisseria gonorrhoeae, Enterobacter aerogenes, and Klebsiella pneumonia.17
Studies have focused on the outer membrane protein F (OmpF) porin because of its well-understood structural and functional properties relative to other major porins. Many studies of changes in porin expression have implicated both OmpF and OmpC. The roles of minor porins, such as NmpC, or those expressed in specific conditions, such as PhoE, perhaps should not be underestimated, but there are far fewer studies on the involvement of these porins in antibiotic resistance. Still, it appears that PhoE can serve as a conduit for entry of the β-lactams, and it may be an even better conduit than OmpF and OmpC if the drug bears a negative charge.18 This may be true as well for chloramphenicol and tetracycline.19
CONCLUSIONS
The rise of bacterial resistance has become a concern in the fight against these pathogens. With little activity in the development of novel antibiotics, the processes of monitoring antimicrobial resistance patterns and wisely using current antibiotics are imperative.
Improper use of antibiotics can lead to the emergence of resistance in a short period of time.20 It is important for clinicians to recognize that, with no control over the development of bacterial resistance, irresponsible or excessive use of the most recently developed antibiotics conveys significant risk. n
Mel Friedman, OD
• private practice at For Your Eyes Only in Memphis
• dfried007@aol.com
Agustin L. Gonzalez, OD
• optometric glaucoma specialist and therapeutic optometrist in practice with Eye & Vision in Richardson, Texas
• ag@txeyedr.com
1. Michigan State University. Antimicrobial Resistance Learning Site. http://amrls.cvm.msu.edu/microbiology/bacterial-resistance-strategies/introductio. Accessed January 14, 2015.
2. Drlica KS, Perlin DS. Antibiotic Resistance: Understanding and Responding to an Emerging Crisis. Saddle River, NJ: FT Press; 2011:12-80.
3. Sköld O. Antibiotics and Antibiotic Resistance. Hoboken, NJ: John Wiley and Sons; 2011:165-186.
4. Crumplin GC, Odell M. Development of resistance to ofloxacin. Drugs. 1987;34(suppl 1):S1-S8.
5. Martinez JL, Baquero F. Mutation frequencies and antibiotic resistance. Antimicrob Agents Chemother. 2000;44:1771-1777.
6. Palmer KL, Kos VN, Gilmore MS. Horizontal gene transfer and the genomics of enterococcal antibiotic resistance. Curr Opin Microbiol. 2010;13:632-639.
7. Hegstad K, Mikalsen T, Coque TM, et al. Mobile genetic elements and their contribution to the emergence of antimicrobial resistant Enterococcus faecalis and Enterococcus faecium. Clin Microbiol Infect. 2010;16:541-554.
8. Jeters RT, Wang GR, Moon K, et al. Tetracycline-associated transcriptional regulation of transfer genes of the Bacteroides conjugative transposon CTnDOT. J Bacteriol. 2009;191:6374-6382.
9. Ochiai K, Yamanaka T, Kimura K, Sawada O. [Inheritance of drug resistance (and its transfer) between Shigella strains and between Shigella and E. coli strains]. Nippon Iji Shinpo. 1959;1861:34-36.
10. Guilfoile P, Alcamo E. Antibiotic-Resistant Bacteria. New York, NY: Infobase Publishing; 2009:38-67.
11. Gualerzi CO, Brandi L, Fabbretti A, Pon CL. Antibiotics: Targets, Mechanisms and Resistance. Hoboken, NJ: John Wiley and Sons; 2014:73-101.
12. Vester V, Douthweite S. Macrolide resistance conferred by base substitution in 23S rRNA. http://aac.asm.org/content/45/1/1.short. Accessed January 29, 2015.
13. Wax RG, Lewis K, Salyers, Taber HA. Bacterial Resistance to Antimicrobials. Boca Raton, FL: Taylor & Francis Group; 2008: 103-132, 133-168, 169-182.
14. VanMeter KC, Hubert RJ, VanMeter WG. Microbiology for the Healthcare Professional. St. Louis, MO: Mosby Elsevier; 2010:404-422.
15. Delcour AH. Outer membrane permeability and antibiotic resistance. Biochim Biophys Acta. 2009;1794:808-816.
16. Nikaido H. Molecular basis of bacterial outer membrane permeability revisited. Microbiol Mol Biol Rev. 2003;67:593-656.
17. Hancock RE, Bell A. Antibiotic uptake into gram-negative bacteria. Eur J Clin Microbiol Infect Dis. 1988;7:713-720.
18. Nikaido H, Rosenberg EY, Foulds J. Porin channels in Escherichia coli: studies with beta-lactams in intact cells.
J Bacteriol. 1983;153:232-240.
19. Mortimer PG, Piddock LJ. The accumulation of five antibacterial agents in porin-deficient mutants of Escherichia coli. J Antimicrob Chemother. 1993;32:195-213.
20. Spatuzza A. Antibiotics: Are We Killing the Cures? Perspectives in Health Magazine. 2002;7:1.